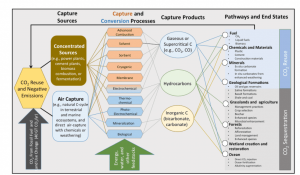
Figure 1. A whole systems approach involving sequestration and re-cycling of CO2 at the Gt/y scale to enable a negative CO2 emission landscape.[1]
At the outset, it is important to put the magnitude of this task in perspective. While a gigatonne of CO2 sounds like a lot of CO2, it only represents about 2.5% of current global emissions of around 40 gigatonnes per annum. Moreover, the emissions are distributed amongst various sources and locations around the world, so no one nation or system can solve this problem at the global level.
In the scenario of atmospheric CO2 concentrations rising to dangerous levels, negative emission CO2 utilization technologies could prove to be one of few known ways to avoid reaching a tipping point of irreversible catastrophic climate change consequences.
In this context, the Figure 1 embodies a ‘whole systems’ view of a negative CO2 utilization landscape, encapsulating all the cross-cutting chemistry and engineering options available today for achieving this lofty goal. The network of options begins with different CO2 capture sources, passes through various capture and conversion processes to diverse repositories and myriad products that include chemicals, materials, polymers, minerals and fuels.[1]
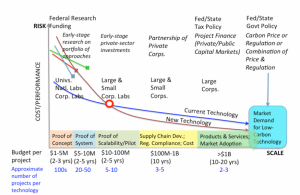
Figure 2. Gt/y CO2 utilization techno-economic learning curve. Note that new technologies that are superior in their performance and capability compared to current ones, could be the preferred technology based on cost, [1].
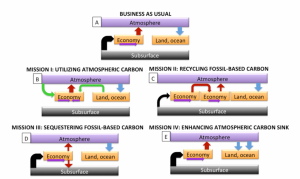
Figure 3. Illustration of schemes to transition a fossil intense to fossil free economy.[1]
This task will not be easy, quick or cheap. Lab-to-market-to-profit at the Gt/y scale has to surmount many hurdles that include science and engineering, demonstration to pilot scales, economics and regulations, supply chains and infrastructure development, business, risk and market research, and policy decisions at the city, regional, national levels. This is a 10-20-year journey, starting with million-dollar R&D investments and ending up having to finance the billion-dollar costs required for market adoption of products and services. The various stages of the techno-economic learning curve that illustrate these considerations are shown in the Figure 2.
Four different deployable approaches, denoted mission I-IV, envisioned to reduce CO2 levels in the atmosphere, compared to a business-as-usual scenario, are encapsulated in Figure 3. The first utilizes atmospheric or land CO2, the second recycles CO2 from combustion, the third does not allow CO2 into the atmosphere, and the fourth boosts land and ocean sinks. Each of these approaches forms one of the pathways in the network of the ‘whole systems’ approach for achieving Gt/y utilization of CO2. It is possible to improve the effectiveness of these individual approaches to Gt/y CO2 utilization by combining them together into more complex systems that could outperform the individual ones.[1]
It’s the Real Thing – Modular CO2 Refineries
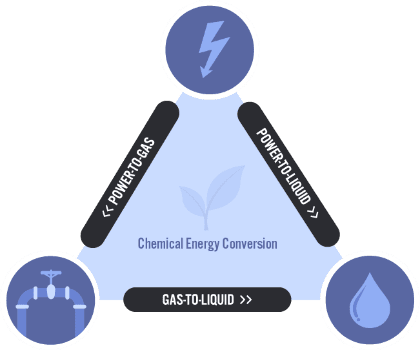
Figure 4. Chemical energy conversion processes.[2]
They all use renewable solar or wind electrical energy to drive (electro)-catalytic conversions whereby the electrical energy is stored in the form of chemical energy using the following processes:
- H2 from H2O by high efficiency steam electrolysis,
- synthesis gas H2/CO from CH4 sources or from CO2/H2 by reverse water gas shift,
- CH4, CH3OH or CnH2n+2 from CO2/H2 or CO2/CO/H2 or CO/H2 by methanation, methanol synthesis or Fischer Tropsch chemistry.
A spin-off company Ineratec (Innovative Chemical Reactor Technologies) birthed from research at Karlsruhe Institute of Technology, KIT, has recently commercialized innovative, compact and containerized chemical plants, which enable all of the above processes.[2]
They are modular CO2 refineries that convert CH4, CO2, H2O and H2 into gaseous and liquid fuels. The mandate of the company is to design, engineer, construct, commission and maintain the modules.
Their modular design enables Lego like construction of CO2 refineries over multiple length scales, from small units to industrial plants.
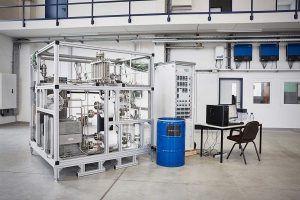
Figure 5. Mobile synthesis unit for a compact containerized CO2 to fuel conversion plant.[2]
The Gas-to-Liquid process operates in two-stages, the first of which involves air oxidation of CH4 into synthesis gas, sourced more often these days from biogas, sewage gas, or landfill gas. In the second step, the synthesis gas is converted into hydrocarbons by the Fischer-Tropsch synthesis, Figure 6. The Power-to-Gas process makes CH4 from CO/CO2/H2 mixtures, which can advantageously be transported in existing pipeline infrastructure for use as a fuel, Figure 6. In the Power-to-Liquid process, similar to Power-to-Gas, the CO2/H2 is converted to synthesis gas, which is then used in the Fischer-Tropsch process to make synthetic fuels, Figure 6. At the time of writing, the aforementioned vision more-or-less delineates a roadmap for the development, implementation and commercialization of the most promising CO2 utilization technologies.
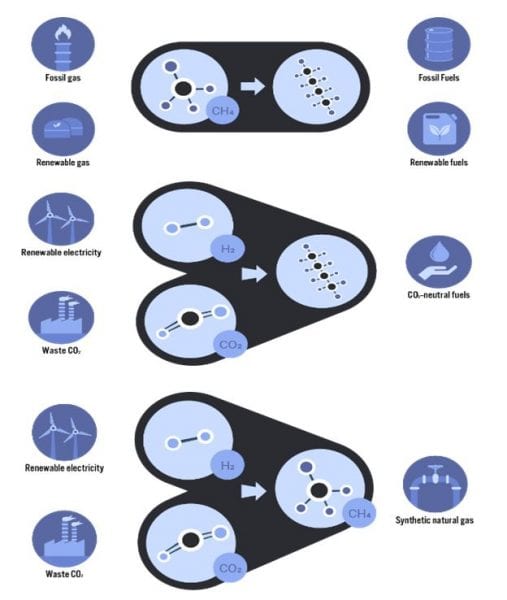
Figure 6. Gas-to-Liquid, Power-to-Gas, Power-to-Liquid processes.[2]
These will all have to be developed and implemented in order to reduce to practice CO2 utilization technologies that offer the potential to reduce CO2 emissions and/or introduce negative emissions at the Gt/y scale.
A pragmatic and fast solution to the Gt/y CO2 utilization challenge will require a systems-based approach that integrates the i) science and engineering, ii) economic and political, iii) public, private and industry expertise of all stakeholders. The overriding goal of this holistic approach is to ensure that all stakeholders are meaningfully engaged in the portfolio of early to late stage technology development to minimize risk and maximize the opportunities for successful implementation of the plan.
Putting it all Together – Energy System of the Future
In 2015, a landmark investment of $329B in the global renewable energy industry, for the first time, surpassed that of fossil fuels. It seems the envisioned transition in our energy system, from non-renewable to renewable, is unstoppable.
Now we must now rise to the challenges of integrating a temporally fluctuating solar and wind renewable energy supply into a smart and secure energy infrastructure. An integrated system of this kind must be especially smart, to be able to generate, store, convert, control, regulate, transport and distribute a wide variety of renewable intermittent energy sources.
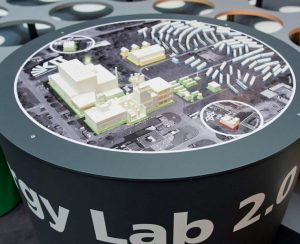
Figure 7. Energy Lab 2.0.[3]
Success in this endeavor is pivotal for enabling the transition from fossil to renewable energy supplies, in a smart grid system that has flexible fuels and loads, temporal fluctuations and geographical variations, in their provision and consumption of energy. For this energy system to generate, store and deliver power-to-heat, power-to-gas and power-to-fuel capabilities, it is necessary to cleverly interconnect and control, renewable forms of electricity, heat, gas and fuels for specific purposes.
The envisioned system will employ solar and wind electricity to produce gas and fuels from the catalysed reactions of H2 with CO2 and/or CO, where the H2 will come from H2O electrolysis, CO2 from renewable sources, and CO-H2 from biomass. Renewable electricity in this network of energy carriers will also be generated using gas turbines able to handle flexible fuels and varying loads.[3]
Decentralized CO2 Refineries built from Modular Transportable Plants
The futuristic vision of a fleet of modular C2 refineries, powered by renewable forms of electricity, built from truck-size containers, transported, delivered and integrated on-site, to industries that have elected to convert their greenhouse gas emissions into synthetic fuels or easily transported liquid fuel precursors, is absolutely brilliant. This connects to the concept of mobile process equipment containers recently developed by chemical industry, Figure 8. Note that the container in Figure 8 is the mobile research lab whereas the real production containers making up the modular refinery would probably look different, as it would not need some of the features of the research lab container.
Development of the envisioned portable, versatile, self-contained, modular synthesis, testing and control systems will enable renewable heat, electrical or solar energy to be used at different industrial sites, to locally capture and purify carbon dioxide and generate hydrogen from water electrolysis. The subsequent conversion of the carbon dioxide and hydrogen into synthetic fuels, provides a novel and exciting paradigm for reducing greenhouse gas emissions, curbing global warming and ameliorating climate change.
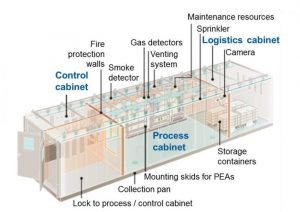
Figure 8. Transportable compartmentalized research container for validation of processes for converting carbon dioxide and water and renewable energy into synthetic fuels, on-site at greenhouse gas emitting industries.[4]
The transportable compartmentalized research container illustrated in Figure 8, modeled after Evonik’s EcoTrainer, a complete self-contained chemical production complex on wheels,[4] brings mobility, flexibility and versatility to Energy Lab 2.0 under construction at Karlsruhe Institute of Technology. Both of these technologies will prove to be key enabling components of the Energy Revolution, well underway in Germany.[3.4]
Imagine an industrial assembly line, manufacturing hundreds of thousands of these mobile CO2 processing containers, delivering and installing them into fossil powered industries all around the world, all tasked to efficiently and economically convert their greenhouse gas emissions into custom-made synthetic fuels.
We are “All” in it Together
It’s a tall order for society but let’s face it, we are all in this world together and we must all now shoulder the Herculean responsibilities of caring for our Earth with the same degree of concern as we care for ourselves and our collective future. If we all work together, we can make it work and we can sustain all life on earth, as we know it today.
Featured graphic courtesy of Chenxi Qian.
References:
- google.de/search?q=SEAB+Task+Force+Report+on+CO2+Utilization+and+Negative+Emissions+Technologies&ie=utf-8&oe=utf-8&gws_rd=cr&ei=ehlnWeqyHYHaU_35rPgF
- ineratec.de/?lang=en
- elab2.kit.edu/english/index.php
- corporate.evonik.de/en/products/product-stories/Pages/chemistry-on-wheels.aspx
Acknowledgements
The numerous fruitful discussions with Professor Roland Dittmeyer of Karlsruhe Institute of Technology about the contents of this article are deeply appreciated.